Antacids for the Sea: Artificial Ocean Alkalinization
A potential tool for adaptation and carbon removal, but more research is needed.
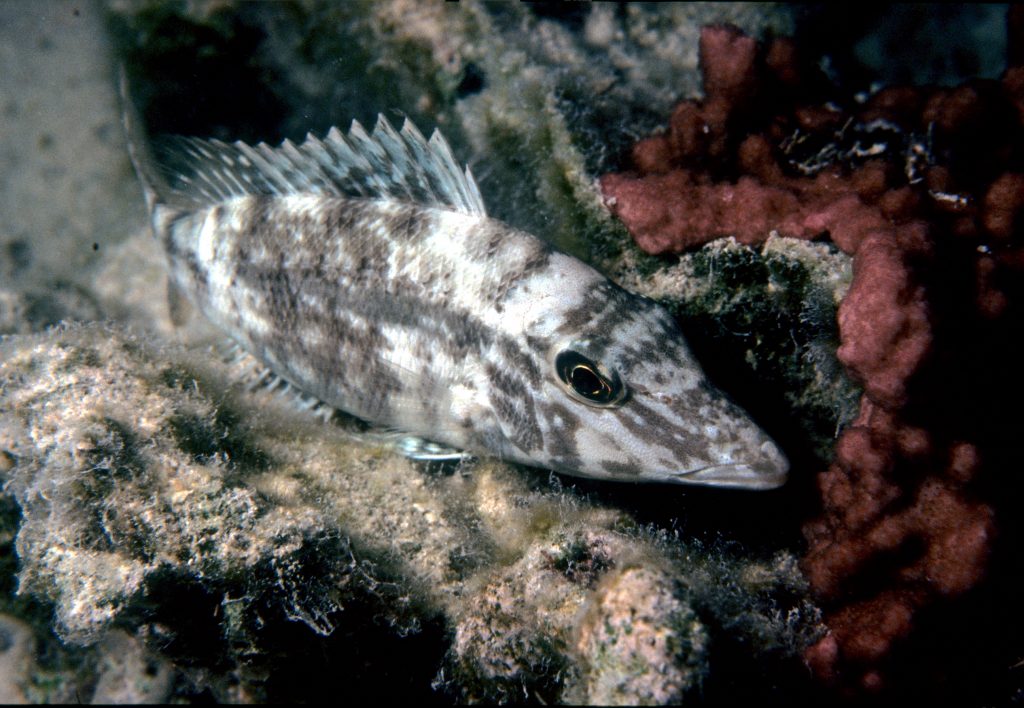
The carbonate cycle helps make the oceans one of the largest carbon sinks on the planet. As the oceans’ surface waters mingle with the open air, they absorb enormous amounts of carbon dioxide (CO2), storing it in the water as carbonic acid and carbonates and as limestone on the seafloor. The carbonate cycle is a natural process that far predates humans and industrialization, but as humans have added more CO2 to the atmosphere, the oceans have absorbed more CO2—more than a third of anthropogenic CO2 emissions released over the past 200 years. Though this absorption has spared us worse climate impacts, it has made the oceans 30% more acidic, on average. Ocean acidification is extremely harmful to marine life, threatening entire ecosystems and the human activities that rely on them.
Some scientists have proposed artificial ocean alkalinization (“AOA”) as a method for countering acidification and increasing ocean carbon uptake. AOA would be a little bit like Tums or Rolaids for the sea. Large amounts of alkaline minerals, such as basalt or olivine, would be crushed up and deposited into the ocean. This would increase the surface water’s total alkalinity, creating a buffer that would cause the oceans to resist increases in their acidity, while also inducing more CO2 absorption. AOA thus has potential as a tool for carbon removal and adaptation, depending on the scale of CO2 sequestration and its impacts on local marine environments.
AOA is part of a set of speculative projects grouped under the name of geoengineering. Geoengineering projects are a dissimilar, eclectic bunch, but are united in their audacity—the idea that humans can find and pull human-sized levers within the planet’s climate system to limit, or perhaps eliminate, the havoc unleashed through the carbon cycle. This blogpost describes how AOA would work, its potential environmental risks, and the big questions looming over research and potential deployment. It closes with a call for moderate, incremental exploration of AOA, primarily as an adaptation measure with CO2 sequestration co-benefits. As technical understanding of AOA matures through research, so too would its governance structures (hopefully). Future policymakers would then be better positioned to decide whether AOA should be pursued more intensively as a negative CO2 practice, or limit its use as a tool to reduce local acidification impacts.
Artificial Ocean Alkalinization and Its Environmental Risks
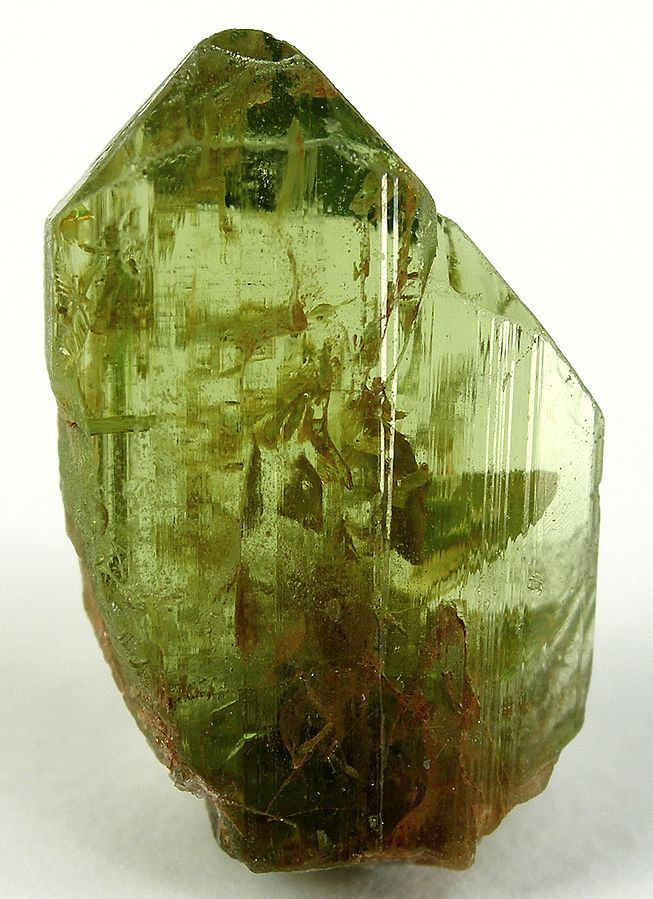
AOA would take advantage of the carbonate cycle’s workings to spur increased CO2 absorption and resist acidification. (A bonus explainer at the end of this post goes into this chemistry in greater detail.) Early research lends support to the proposal—field research on the Southern Great Barrier Reef has shown that increasing ocean total alkalinity to pre-industrial levels can spur growth in coral reef. The results of this study suggest that AOA could be used as an ecosystem adaptation measure, depending on how cost-effective treatment is, as well as the balance of ecological benefits and harms.
Deploying AOA as a large-scale measure for carbon removal, on the other hand, would entail significantly greater environmental risks. Such a project would involve depositing many thousands of tons of pulverized material into the ocean, with the direct purpose of altering ocean chemistry over enormous sections of the sea. The potential impacts to ecosystems could be large, though they would vary depending on the size and location of alkaline deposition, ecosystem sensitivity, and the extent to which depositions migrate from treatment areas or precipitate out of the water. There would also be significant environmental impacts on land: implementing AOA as a carbon removal method would require expansive operations to mine alkaline materials, grind them into sufficiently small grains, and then transport them to ships for deposition.
(I use the word “deposit” instead of “dump” because “dumping” is something of a term of art within the Law of the Sea; for those interested, here’s an in-depth legal analysis of international anti-dumping law and one episode of marine-based geoengineering research.)
Regulating Artificial Ocean Alkalinization Projects
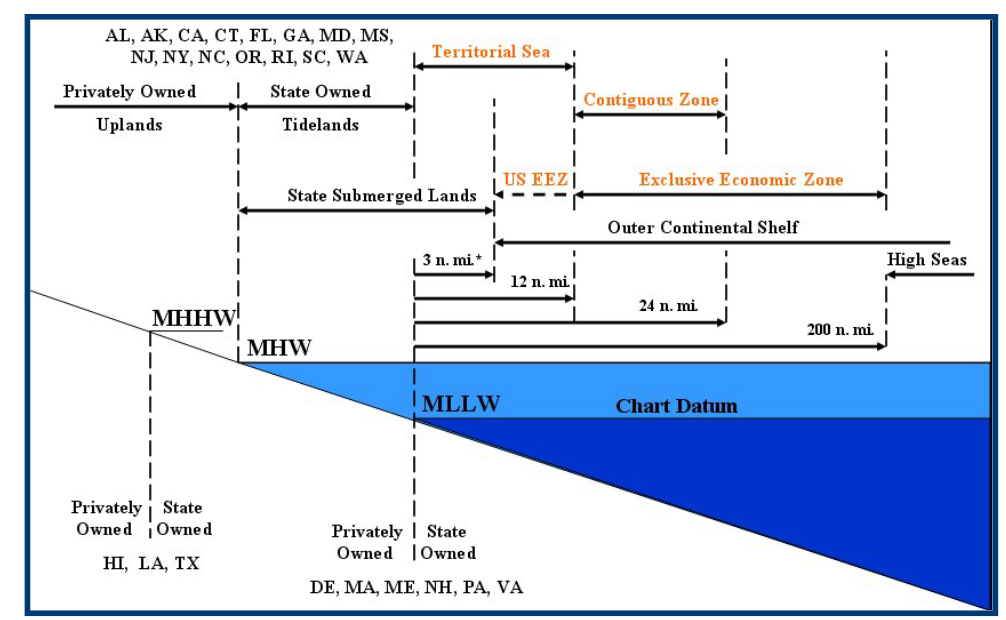
AOA Research
Some studies have suggested that AOA would be more effective if deployed in coastal waters rather than in deeper waters further from the shore. This has legal significance, as international law divides jurisdiction over oceans into zones. Under the U.N. Convention on the Law of the Sea, coastal countries possess sovereignty over the water, air, seabeds, and marine resources within their territorial sea – the part of the ocean within 12 nautical miles of the coastline. If research were to be carried out in coastal waters, it would likely fall within the territorial sea, making the activities subject to the jurisdiction of the relevant coastal country.
We can imagine such experiments following the permitting and environmental assessments of equivalently impactful research efforts currently undertaken in coastal waters. If research were to occur in U.S. coastal waters, this would mean satisfying the regulatory processes of the state and federal government. The environmental impacts arising from depositing small amounts of alkaline materials do not seem so dissimilar from the risks of other experiments as to warrant distinct, geoengineering-specific analysis. This seems especially true where the research is designed to explore alkalinization as an adaptation measure.
Jurisdiction over marine research becomes somewhat more complex within a country’s exclusive economic zone (“EEZ”), the area of the ocean beyond the territorial sea, up to 200 nautical miles from the coastline. Though a country has less control over activities in its EEZ than in its territorial sea, it would still be authorized to regulate research activities so as to limit harms to marine resources. This means that if “coastal waters,” for the purpose of AOA research, falls within the EEZ, the relevant coastal country would still have considerable authority to regulate those activities.
Because the oceans are divided between many countries and many jurisdictions, there is a risk of researchers “shopping” for coastal waters with less regulation. It may be wise, then, to develop additional international governance tools to ensure that AOA research complies with acceptable environmental and scientific standards. Instruments developed for ocean iron fertilization (“OIF”) research might provide a useful starting point, as the two technologies resemble one another: both would involve introducing foreign materials to the ocean to alter its chemistry to induce carbon uptake. AOA governance, however, would need to take account of its distinct value as an adaptation measure, as well as the fact that it would not operate directly through “ecological disruption,” as would be the case with OIF.
AOA Deployment
Governance questions become more challenging when considering deployment of AOA on a climatically significant scale. An AOA program of such size would raise several concerns under international environmental law. A few key concerns:
- Uncertainty: What are the risks of harm to marine ecosystems? What are the risks of transboundary harm? Which risks and harms are “tolerable”?
- Control: Who decides whether to deploy AOA? How would the decision be made? Who resolves disputes?
- Liability: Which injuries are compensable? What is needed to show attribution? What remedies are available? Who bears the burden of repayment?
Our ability to begin to answer these questions is limited by our ignorance of AOA’s impacts on ocean chemistry, ecosystems, and the carbon cycle. Further research could reduce our ignorance in these areas, but may fail to resolve, or even increase, uncertainty over risks.
Given the widespread aversion to invasive geoengineering techniques, a less radical path appears more likely. Further research should continue to develop AOA as a local adaptation method, regulated primarily under the domestic law of coastal countries. If carbon sequestration proves to be measurable, long-lasting, and net negative, CO2 removal could become a co-benefit of AOA adaptation programs. Perhaps these CO2 withdrawals could be incorporated within a country’s nationally determined contributions under the Paris Agreement; that would depend on their size and the willingness of the international community to recognize such unorthodox withdrawals.
Experience with localized AOA may encourage greater depositions to capture more carbon. It might not. But this moderate path—gradual exploration of AOA as an adaption measure, with a potential carbon-removal co-benefit—would allow international governance structures to grow as technical knowledge of process matures. Future policymakers would then be well-positioned to determine whether to scale up AOA further as a carbon removal technique: the risks, rewards, and uncertainties of the enterprise would be better understood.
(Many thanks to Dr. Shuchi Talati for help with fact-checking and comments. All mistakes are my own.)
***
Bonus Section: A Very Basic Explainer on Ocean-Carbon Chemistry
The following gives a simple explanation of pH, total alkalinity, and the ocean carbonate cycle. It’s for the readers out there who, like me, have little background in the sciences but still would like to try to understand ocean chemistry.
Acids, bases, and pH
Most of us are familiar with the pH scale and the litmus strips used to measure it. The term “pH” stands for “power of hydrogen,” and represents the concentration of hydrogen ions (H+) in a solution. (Ion describes a particle that carries a negative or positive charge.) When added to a solution, acids will release hydrogen ions, while bases will “consume” hydrogen ions. The more hydrogen ions in a solution, the lower a solution’s pH, and the more acidic it will be. Conversely, a more basic solution has fewer hydrogen ions and a higher pH.
If that seems backward, it’s because pH is measured on a negative logarithmic scale (-log(H+)). The Richter scale, used to measure earthquakes, is another logarithmic scale. As all Southern Californians know, seemingly small changes in Richter scale readings can represent dramatic changes in earthquake intensity. So too with ocean pH—the decrease in average ocean pH observed since the beginning of human industrialization, from about 8.2 to about 8.11, represents a 30% increase in ocean acidity. The oceans are still slightly basic, but are becoming less so and at a faster rate as humans continue to emit CO2 into the atmosphere.
Total alkalinity and the carbonate cycle
Alkaline is sometimes used as a synonym for basic: an alkaline solution, like bleach, has a much higher pH than, say, lemon juice. Total alkalinity, on the other hand, describes the ability of a solution to resist changes in its pH despite the introduction of acids or bases. Essentially, a stew of positive- and negative-charged ions within a solution form a buffer that offsets reactions which would otherwise change total pH. (This is why “total alkalinity” is sometimes described as “buffering capacity.”) Oceans have a very high total alkalinity—their day-to-day pH levels remain relatively constant despite continuous interactions with the open air. This is why we can enjoy a day at the beach without worrying about getting a chemical burn; total alkalinity prevents rapid dramatic changes in pH.
The ocean’s total alkalinity is informed by the carbonate cycle, which is a set of interactions between water and air that draws CO2 out of the atmosphere. As air comes into contact with the ocean, some of its CO2 is absorbed, forming carbonic acid (H2CO3). Carbonic acid is a very weak acid, which means the chain of reactions it kicks off in the ocean are determined by a state of equilibrium within the water and air. (“Strong” acids, like hydrochloric acid, on the other hand, will completely disassociate.)
Following this equilibrium, some of the carbonic acid (H2CO3), but not all, will disassociate, losing a hydrogen ion (H+) to the sea to become bicarbonate ions (HCO3–). Some of those bicarbonate ions, but not all, will disassociate further, shedding another hydrogen ion (H+) to become carbonate ions (CO32-). Finally, marine lifeforms, like corals and mollusks, will bind some of those carbonate ions (but not all!) to calcium, forming calcium carbonate (CaCO3)— the building block of shells. When those lifeforms die, their shells gently fall down to the seafloor, forming limestone. The ocean carbonate cycle therefore looks something like this:
Carbon dioxide + water ⇌ carbonic acid ⇌ bicarbonate ions + hydrogen ions ⇌ carbonate ions + hydrogen ions (with some carbonate falling out as shells)
Again, the carbonate cycle is powered by an equilibrium series of reactions (“⇌”) because carbonic acid is a weak acid: only some of the carbonic acid, bicarbonate, and carbonate at each stage disassociates. The “flow” of reactions within the carbonate cycle depends on several conditions up and down the chain, such as atmospheric CO2 concentration, ocean pH (H+ concentration), water temperature, and pressure. Changing one condition will thus cause changes up and down the equilibrium chain.
For example, higher concentrations of atmospheric CO2 spurs greater absorption of CO2 by the ocean, leading to higher levels of acidity. Higher acidity means there are more hydrogen ions in the water, and some of those additional hydrogen ions will bind with carbonate ions to form bicarbonate, leaving less carbonate available for mollusks to use in their shells. (This is why ocean acidification has the potential to be so disastrous: marine shells can literally dissolve away as acidity rises.) AOA would be another example of chemical change rippling through the carbonate cycle. Adding pulverized alkaline minerals to the sea causes chemical reactions that consume hydrogen ions in the water. Because the carbonate cycle’s flow of reactions “likes” to stay in equilibrium, the water will pull more CO2 out of the atmosphere to produce more carbonate ions and hydrogen ions.
Reader Comments
One Reply to “Antacids for the Sea: Artificial Ocean Alkalinization”
Comments are closed.
An alternative approach would be removal or sequestration of CO2 with artificial carbonate cycles at the source (e.g., fossil fuel power plants).
The ocean carbonate cycle works to sequester carbon because organisms such as phytoplankton convert CO2 into calcium carbonate shells, which sink to the ocean floor when the organisms die.
In recent years, experiments with sea urchins have discovered that nickel serves as a catalsyt in their shell formation, and that nickel nanoparticles can remove CO2 from water to form calcicum carbonate.
This presents the possibility of removing or sequestering CO2 at the source by controlling emissions from fossil fuel power plants with artificial carbonate cycles on site (which may involve nickel nanoparticles or other technologies), rather than acidifying and then alkalizing the oceans.