The Fuss about Methane
Part 1: Science and weird facts
Methane is getting a lot of attention in climate debates. There was even a “Methane Day” last Tuesday at the climate conference in Glasgow. Several new regulations controlling methane emissions have been adopted recently, including two new rules for the US oil and gas sector announced last week. There’s a new informal international agreement to limit methane emissions, and a still-unresolved effort to put a charge on methane emissions into the forthcoming reconciliation bill. And more methane initiatives are surely on the way.
There are several good reasons for this. Methane is essential to control, since stabilizing climate requires reducing all anthropogenic greenhouse-gas emissions to net-zero. Methane is a pretty big contributor to heating, second only to CO2. Moreover, for reasons I’ll explain below, cutting methane brings especially strong benefits over the next few decades. There are even indications that near-term cuts might be easier to achieve for methane than for CO2, for a mix of technical, economic, and political reasons. None of this means methane controls can replace CO2 controls; but it does make methane an especially attractive candidate for immediate and steep cuts.
This post is an introduction to methane in climate change: where it comes from, how it’s different from CO2, how those differences matter, and what that all means for controls. I won’t go into details on the current state of methane controls and proposals for new ones. That’s for a subsequent post.
Background: More science than you want
Methane, chemically CH4, is the smallest and simplest hydrocarbon. “Hydrocarbon” means just what it sounds like: molecules containing only hydrogen and carbon. Other familiar hydrocarbons include the propane (C3H8) in the tanks at your off-grid survivalist cabin, the butane (C4H10) in your cigarette lighter and camp stove, and the non-specific mix of hexanes through octanes (similar molecules containing six to eight carbon atoms) in your car’s tank, which you call “gasoline.” The lightest hydrocarbons, like methane, are gases at room temperature, so they’re either delivered through pipes or compressed into tanks. As you move from smaller to larger hydrocarbon molecules, you first get light, volatile liquids (Aah, the small of gasoline), then increasingly heavy and sludgy liquids (diesel fuel, kerosene, heating fuel oil, marine bunker fuel), then soft spreadable solids (Ever wonder why Vaseline is called “petroleum jelly”?), then more solid solids like paraffin.
We most often encounter methane as the largest component of natural gas: methane makes up 75 – 95% of the gas coming to your stove and furnace.
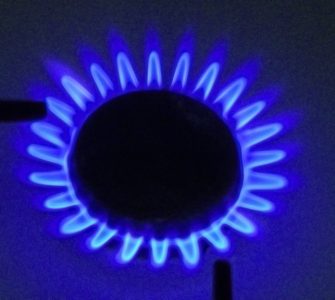
“Natural gas” was a marketing term for this then-new, safer gas pumped through urban distribution pipes starting in the 1940s. It replaced an earlier, more dangerous, product called “town gas” or “coal gas,” which consisted mainly of hydrogen and carbon monoxide and was produced by heating coal in
the absence of air. Compared to town gas, natural gas has the great advantage that it’s not toxic: that’s why no one kills themselves by sticking their head inside an (unlit) oven in literature or film set more recently than the 1950s. Natural gas also has a higher energy content, so your kettle boils faster – but these benefits come at the cost of a greater explosion risk. Fires and explosions from leaky pipes were fairly common as the new gas was rolled out through distribution systems in the 1940s and 1950s, until the utilities got better at controlling leaks – although as we’ll see below, this improvement was enough to limit local explosion risk but not enough to control methane’s contribution to climate change. A big early challenge to effective leak control was that methane and the other constituents of natural gas are all odorless, so you can’t smell leaks. To solve this problem, utilities came up with the clever innovation of putting a little bit of stinky stuff into the gas, so people reliably report leaks. This stinky stuff is a blend of reduced sulfur compounds, which all have strong smells, although it doesn’t include the best-known of these stinky reduced-sulfur compounds, Hydrogen sulfide or rotten-egg gas (H2S), because of its high toxicity. The odorant is usually a mixture of dimethyl sulfide, tetrahydrothiophene, and various mercaptans. Different utilities use different mixes, aiming to make the smell really strong, but not quite like anything else: a hint of skunk, but not too much; a little bit of kerosene; a smidgen of rotten eggs; etc. When I first learned about this, I wondered (and still wonder) about who does that job: in particular, are they perfumiers who didn’t make it in the big leagues? “Marcel, je regrette to inform you that your nez, he is too crude for Chanel, so it’s off to Gaz de France for you!”
Almost all the methane on Earth is formed in two ways. First, by chemical conversion of organic material at high temperature and pressure deep underground: that’s how most of the methane in natural gas is formed. Second, by microbial respiration in anoxic environments. The clever microbes that do this, mostly Archaea (i.e., not plants, animals, fungi, or bacteria), get their energy by breaking down organic molecules via a different chemical pathway than we air-breathers use to get our energy, which does not require oxygen and ends in methane instead of carbon dioxide and water. This anaerobic respiration has to take place where there is no oxygen, so it mainly happens in two places on Earth. First, underwater – in sediments on the bottom of swamps, lakes, and the ocean. And second, in the guts of animals – termites, cows and other ruminants, and us. We’re not a big source – even with legume-heavy diets, the cows emit much more than we do – but I mention it to honor the amusement (rude to be sure, but only slightly dangerous) practiced since time immemorial by adolescents brought up as badly as me.
OK, on to methane in the environment: The headline here – whether you’re talking about atmospheric concentrations, climate impacts, or emissions – is that there is a lot less methane than CO2, but it’s a more potent climate heater and it’s increasing faster.
Atmospheric concentrations
CO2 is at about 410 parts per million by volume (ppm) in the air, about 50% higher than before the start of large-scale fossil-fuel use. Methane is at about 1,890 parts per billion by volume (ppb) or 1.89 ppm, but that level is about 2.6 times the pre-industrial concentration. Methane’s concentration increases have been more variable over time than those of CO2, and these variations are not fully understood. For example, methane stayed roughly flat for about a decade from the late 1990s, but has increased rapidly since then – by nearly 16 ppb in 2020 over 2019.
Climate impact
That small concentration of methane in the atmosphere makes an outsized contribution to global heating. Human-source atmospheric methane now adds slightly less than 1 watt per square meter (W/m2) of radiative forcing, versus 1.7 W/m2 from elevated CO2. Or in terms of temperature effect, anthropogenic methane accounts for about 0.5°C of the global heating already realized, CO2 for about 0.75°C. (Note: you might find these figures confusing, since total heating is only about 1.2°C. The issue is that total human radiative forcing includes several parts that heat and some that cool, so counting separate heating contributions like this leaving out the cooling parts gives too much heating.)
Emissions
Here too, CO2 is much bigger, but methane is growing faster and punches above its weight. Anthropogenic emissions of CO2 are about 36 billion tons per year (GtCO2/yr), while methane emissions are several hundred million tons per year (MtCH4/yr). The most recent comprehensive estimate is that worldwide methane emissions from all sources are about 570 Mt/yr (range ~ 550-600), of which about 60% (360 Mt) come from human sources, the other 40% from natural sources. Of that human-source share, about 35% comes from fossil-fuel production, processing and use (oil and gas 23%, coal mining 12%); 40% comes from agriculture (livestock 32%, flooded rice fields 8%), and 20% comes from waste, mostly landfills (because they are packed so tight that air can’t get in) and wastewater. The natural emissions are mostly from wetlands (about 85%), the rest from termites, wild ruminants, and a few miscellaneous sources.
There is a fair amount of uncertainty in these emissions budgets. These numbers are “top-down” estimates – inferred from observing how atmospheric concentrations vary over time and location. By contrast, “bottom-up” estimates observe the operating levels of activities that emit methane, measure emissions from a sample of these – this natural-gas field, this feedlot, this dairy operation – then assume the emissions-to-activity ratios measured at those sources apply to the whole sector. Bottom-up emissions estimates are presently about 30% higher than top-down estimates, but the latter are considered more reliable. There are significant uncertainties in anthropogenic sources, but the largest uncertainties are for natural emissions, especially wetlands.
Uncertainties and Confusions 1: Be careful what (and how) you measure
There are a few persistent sources of uncertainty and confusion about methane, that you run across repeatedly in policy debates and news accounts. The most basic of these – a source of potential confusion, not an uncertainty – concerns how to measure emissions, concentrations, and their effects, in order to provide the basis to compare different greenhouse gases.
There are two issues: do you measure and compare by volume or by mass, and if you measure by mass do you count the mass of the whole molecule or just the carbon part of it? This latter issue is actually a bigger source of confusion for CO2 than for Methane. In the early days of the climate issue, when discussions were mainly scientific, normal practice was to measure CO2
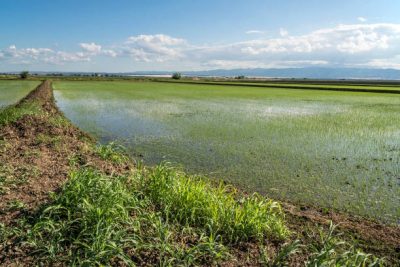
by the mass of only the carbon atom in the molecule, not the whole thing. More recently, as climate change moved into broader public and policy debate, it became standard to measure CO2 by the mass of the whole molecule. Since one carbon atom has a mass of 12 Atomic Mass Units (AMU), while one oxygen atom has mass of about 16 AMU, the total mass of a CO2 molecule (one carbon plus two oxygen atoms) is 44 AMU. Measuring by mass of CO2 is now so standard that even IPCC reports have mostly switched to doing it that way. But that ratio, 44/12 or 3.67, still crops up repeatedly in conversations about CO2 emissions, reduction costs, and policies. So if you see a reported emissions figure that seems much too small, or an emissions price or cost of reduction that seems much too big, it’s probably expressed in tons carbon rather than tons CO2. Careful writers always write units explicitly as tCO2, or (if comparing multiple gases) tCO2e, where the “e” is for equivalent. But not all writers are always careful. The same issue applies to methane, but its effect is numerically smaller. In a methane molecule that mass of the carbon is again 12, while the whole molecule is about 16 because each hydrogen atom is 1 AMU. As with the modern convention for CO2, most reporting of methane counts the mass of the whole molecule and careful writers make that explicit by writing tCH4. But if some figure seems mysteriously off by about a quarter, the first thing to check is whether someone is reporting just the mass of the carbon.
Both measuring by mass and measuring by volume are used regularly, so you have to be careful. Generally speaking, emissions – both methane and CO2 – are reported by mass, but atmospheric abundance is reported by volume, in what is typically called a “mixing ratio.” Measuring by volume is equivalent to counting molecules, since in gases at the same conditions (meaning the same temperature and pressure), volume is proportional to the number of molecules present. If you remember your high school chemistry, this is called Avogadro’s Principle: in words branded on my brain by rote repetition, “equal volumes of any two gases at the same temperature and pressure contain equal numbers of molecules.” This means that one molecule of methane in the atmosphere occupies the same volume as one molecule of CO2, but has less mass by the factor 16/44. So to compare the relative heating contributions of methane and CO2, you have to be clear whether you’re comparing molecule to molecule or ton to ton. The heating contribution of one methane molecule is about 25 times higher than that of one CO2 molecule. But because the mass of that methane molecule is only a little more than one-third that of the CO2 molecule, comparing mass to mass means you’re counting the effect of nearly three times as many methane molecules as CO2 molecules. So on a mass-to-mass basis, methane has a heating contribution about 70 times higher than CO2. Methane also has indirect effects on heating, due to chemical interactions by which methane changes the levels of other greenhouse gases. Including these indirect effects increases methane’s heating effect to 45 times that of CO2 comparing molecule to molecule, or 125 times that of CO2 comparing mass to mass.
Uncertainties and Confusions 2: Isn’t scientific progress exciting?
Scientific knowledge of atmospheric methane has made substantial advances over the past decade and continues to do so, but these advances sometimes generate confusion because numerical estimates of physical quantities or other results of different vintages often get circulated without explaining or even noting the differences.
The most important recent change was a new measurement of methane’s infrared absorption in 2016. Previous estimates had only included methane’s absorption in the thermal infrared region, that spectral region of wavelengths around 8 to 14 microns where most of both the natural greenhouse effect and current human-driven heating happen. But methane also has a couple of absorption bands in the shortwave infrared region, closer to visible light (around 1.65 and 2.3 microns, when visible light is from about 0.4 to 0.7 microns). Including these bands increased methane’s calculated total heating contribution by about 25 percent.
In addition, several recent studies have substantially revised prior estimates of methane’s emissions budget – meaning how much comes form what sources, and where it goes. The single biggest effect of these changes has been to increase estimates of emissions from the oil and gas sector – a change that has occurred in parallel with a big increase in actual emissions due to rapid, fracking-enabled growth of production, especially in the United States . One 2018 study combined on-site and aircraft measurements to estimate US oil and gas sector emissions about 60 percent higher than in the official emissions inventory. This higher figure implied a leakage rate of 2.3% of total US gas production, as opposed to the official estimate of 1.4%. Another study published this year measured methane in the air all around the Boston metropolitan area, aiming to improve estimates of emissions from natural-gas distribution and end-use (which is the major source in a big city that doesn’t have oil or gas wells, refineries, agriculture, or landfills nearby). This study found emissions roughly triple the estimates derived from activity-based inventories, suggesting that leakage rates from the whole system are even higher than found in the 2018 study, from 3.3 to 4.7% of total production.
Many of these advances in understanding methane emissions are coming from more numerous and advanced satellite instruments that are providing increasingly fine-grained coverage in space and time, along with improved models and analytic methods to integrate observations from multiple platforms and sources. In addition to generally increasing estimated emissions, these advances in observational precision are also increasingly showing that very few sources and events, often related to accidents or equipment malfunctions, contribute a much larger than expected share of total emissions. This suggests that fine-grained, continuous emissions monitoring and a rapid response capability are much more important for emissions control than was previously recognized.
Uncertainties and Confusions 3: Wait, how much worse is methane than CO2?
Perhaps the biggest source of confusion in understanding methane’s role in global heating doesn’t much come from scientific uncertainty, but from the intrinsic ambiguity involved in trying to represent in a single number the relative heating effects of two gases with widely different atmospheric lifetimes after they are emitted.
How much more does methane contribute to global climate change than CO2? You hear a shockingly wide range of answers. It’s 7 times stronger. No, it’s 25 times stronger. No, it’s 80 times stronger. No, it’s 125 times stronger. These numbers are all different examples of “global warming potentials” (GWP) – a regulatory metric that defines how to convert emissions of different gases into a common scale, sort of like the exchange rate between two currencies. Any regulatory system that covers multiple gases under a single control mechanism has to specify such an exchange rate, to determine how much credit you get for reducing a ton of one gas, relative to another.
Since GWP’s are all measured relative to CO2, the GWP of CO2 is always one, by definition. For other gases, the GWP depends on two different properties of the gas: how strongly it absorbs infrared radiation; and how long it stays in the atmosphere after it is emitted. Only a little of the wide range in GWP figures comes from changing scientific knowledge over time. Most of it comes from differences in atmospheric lifetime, and crucially, different judgments of how to take account of these atmospheric lifetime. To cut to the chase: methane heats much more strongly than CO2 while it’s present in the atmosphere, but it doesn’t stay long. CO2 has a weaker heating effect but stays around much longer. Relative to CO2, methane lives fast, dies young.
How much a unit of gas contributes to heating while it’s there is measured by its instantaneous radiative forcing, or infrared absorbance: how much energy it absorbs and re-emits per unit mass relative to CO2. This is determined by the gas’s absorption spectrum, and it can be measured at a single moment in time. When I said above that a unit mass of methane heats 70 times more strongly than CO2, or 125 times more strongly including indirect effects, those were comparisons of instantaneous infrared absorbance or instantaneous radiative forcing per unit mass.
But it’s not particularly useful to measure total contributions to heating at a single instant of time, because we care about how hot it will get and getting hotter takes time. Each gas’s total contribution to heating thus also depends on how long it stays in the atmosphere to keep heating once it is emitted: its atmospheric lifetime.
Atmospheric lifetimes don’t operate like an on/off switch. If something has an atmospheric lifetime of 10 years, that doesn’t mean it all stays for ten years then instantly disappears. Rather, atmospheric species are all removed by processes that operate continuously, but at widely different speeds. Atmospheric removal processes typically remove a constant fraction of whatever amount is present in each time interval: ten percent the first year, ten percent of what’s left the second year, and so on. If a species is removed by processes that work this way, its remaining amount decreases exponentially over time, like the decay of a radioactive material. The rate at which any radioactive material decays is described by its “half-life”: the time it takes for any starting amount of the material to decrease by half. It’s characteristics of exponential change, whether growth or decay, that changing by a given factor, in this case reducing by half, takes the same length of time, no matter how much you start with. Atmospheric lifetimes could also be expressed as half-lives, but for computational simplicity they are usually expressed as the time required to decrease, not by a factor of two but by a factor of “e” (an irrational number of about 2.72, the base of the natural logarithms).
This discussion of atmospheric lifetimes is only strictly correct for substances that have just one exponential removal process. Many atmospheric gases are removed by more than one process, operating at different speeds. In this case, atmospheric lifetimes blend the effects of these different loss processes. Methane is removed by a few different processes, but one of them – oxidation by the hydroxyl or OH radical – is the most important, so methane’s atmospheric lifetime is close to its atmospheric lifetime relative to just this loss process. There is, however, one additional wrinkle that affects methane’s atmospheric lifetime. Those hydroxyl radicals are really important, but are also really rare – on order one part in 1018 of air. This concentration is so tiny that researchers usually count OH abundance not as volume ratios, but in terms of the number of molecules present per cubic centimeter of air, with those numbers as low as hundreds or thousands of molecules per cm3. As a result, methane concentrations as low as parts per billion can deplete the OH, so the more methane is present in the air, the longer its atmospheric lifetime: It’s kind of like methane survives longer by overwhelming its predators (in ecology) or flooding the defensive zone (in sports). Researchers handle this wrinkle by separately counting the average lifetime of all methane molecules present in the air (about 8 years), as opposed to the lifetime of an additional bit of methane added to the present amount (about 12.5 years). Because emissions are in fact increasing the amount of methane present, it’s the latter of these lifetimes, 12.5 years, that is relevant for calculating the incremental heating from methane emissions.
Unfortunately, the one atmospheric trace gas to which a simple exponential representation of atmospheric lifetime fits the worst is CO2. It is removed by several processes that operate at vastly different speeds, from weeks to millions of years. For practical purposes, people just think of its lifetime as thousands of years or longer. That complexity doesn’t affect GWP calculations, however, since CO2 is the baseline relative to which the heating effect of other gases is calculated.
OK, we’re ready to calculate GWP. Suppose that at some given starting time, you emit one ton of CO2 and one ton of methane. How much does each of those contribute to global heating? As noted above, right after they’re emitted that ton of methane is heating 125 times more strongly than the ton of CO2. That factor of 125 measures how much harder the methane is pushing the climate to heat up, the instantaneous forcing. But you probably care about atmospheric heating not this week, but over some longer period. As time passes, each gram of methane remaining in the atmosphere keeps pushing just as hard, but fewer grams remain to do that pushing: to stretch the sports metaphors further, each member of the team is just as strong, but there are fewer people left on the team. With an atmospheric lifetime of 12.5 years, only a little more than a third of the original ton emitted remains in the atmosphere after that time, a little more than one-eighth after 25 years, and so on.
The total heating effect of that ton of methane after any time is then the product of that instantaneous forcing per unit, multiplied by the amount of the original ton that remains, added up (or in calculus terms, integrated) from the starting time up until the time you’re looking at. The total heating effect of the initial ton of CO2 is calculated the same way: the heating push per unit mass, multiplied by the mass remaining from the initial one ton emitted, added up (integrated) from the starting time to the time at which you’re measuring. The ratio of these two calculated total heating effects (Ta-da!) is the global warming potential of methane. (Trigger warning: Don’t look at the figure if integral signs scare you.)
There are two things to note about this calculation: First, remember that the GWP of CO2 is set identically equal to 1. That doesn’t mean the heating contribution of CO2 is fixed – it does decrease over time, albeit very slowly – it just means that the heating effect of CO2 is used as the unit of measurement, relative to which all the others are calculated.
Second, and crucially, when you compare two gases with different atmospheric lifetimes, that ratio is going to change a lot depending on the time horizon you use to do the calculation. You could calculate the one-week GWP of methane if you wanted. Since essentially none of the initial ton is removed that fast – a 12.5-year atmospheric life is short, but it’s not that short – this would be nearly the same as the ratio of instantaneous radiative forcings, 125. Over longer periods, the ratio between the cumulative heating effect of methane and CO2 decreases, because the added-up effects over time include more and more time when very little of the initial ton of methane remains, while the fraction of the original CO2 remaining is much closer to constant. So when you do the comparison over 20 years, methane’s heating contribution decreases to about 85 times that of CO. After 100 years, it’s down to 30 times that of CO2, and the further ahead you look, the smaller the ratio gets. What would the GWP of methane be over a million years? Your first guess might be that it’s zero, and that would be entirely reasonable. Over periods that long, the ratio of the two summed (integrated) heating effects is going to be completely dominated by thousands of years over which the original methane emission is essentially gone, while the original CO2 is mostly still there and still heating.
There are a couple more wrinkles, however, that make this intuitively attractive answer not quite right. The original methane emission might be gone, but what happened to it? If the methane went away by the dominant loss process, OH oxidation, then each methane molecule got turned into a CO2 molecule: a much weaker heater than methane, but still a heater. That would suggest that over very long periods, methane’s GWP should converge to 1 rather than zero. But as the newest IPCC assessment report pointed out, there is still one more wrinkle: the really really total heating contribution of methane depends on how the methane got made. If it was formed by anaerobic decomposition of organic matter (those cows and rice fields), then the carbon atom in the methane molecule was removed from the atmosphere recently by photosynthesis, so counting the whole system life-cycle you don’t need count the CO2 molecule that remains after all the methane is oxidized: that carbon atom was taken from atmospheric CO2 by photosynthesis within the past few years, and now it’s back there. But if the methane came from oil, gas, or coal production, then its carbon atom had been stored away from the atmosphere for millions of years, so you do need to account for putting it back. As a result, the new IPCC assessment for the first time reports two separate values for methane’s GWP over each time period: a slightly lower one for biological-source methane, and a slightly higher one for fossil-source methane.
Isn’t this an Environmental Law blog? Putting it all together.
This all leads to a couple of important points for greenhouse-gas control.
First, it’s not possible to answer the question “how much worse is methane,” without specifying the time period over which you’re making the comparison. This should be the time period over which you care about climate effects. If what you care about is limiting global heating in the year 2100, then immediate control of a short-lived gas like methane is not an efficient place to focus your efforts, since current emissions will essentially all be gone by then in any case. But if you are most concerned about reducing global heating over the next few decades, short-lived gases like methane are much higher priorities for control. That ton you cut today would have had very strong heating effect over the next 10-20 years, which can be avoided by cutting the emission now. The GWP quantifies this shift in relative priorities depending on the time-horizon of concern. It is not a scientifically determined quantity: it’s a regulatory parameter that depends on both scientific knowledge, and normative judgments about what time horizon we most care about.
Second, scientific knowledge keeps moving, so understanding of environmental impacts changes over time. That’s a good thing for effective environmental policy, because it means that laws and policies can be based on increasingly accurate understanding of the thing being controlled. But when snapshots of today’s knowledge get written into laws or policies that will stay constant over time – as when GWP estimates from one source today get written into a control that will stay on the books – there is a tension between keeping regulations aligned with the best current scientific understanding, and keeping regulations stable to limit regulatory uncertainty for long-term investments.
This tension is very much alive in using GWPs to regulate. Various regulatory decisions – in different countries, and over time – have taken GWP estimates from some recent study or assessment report, then continued to use them without updating as scientific knowledge changes. The most prominent issue has been a change over time in which duration of GWPs are prioritized. In early days, the focus was on long-term climate control so most actions that used GWPs used a 100-year time horizon. Even the Paris Rulebook specifies 100-year GWPs, although it also authorizes the use of other metrics (i.e., shorter time-horizon GWPs). And of course, since the Paris Agreement does not specify any binding control levels, the choice of metric does not yet directly affect regulations. But over time, as views of climate change have shifted toward regarding it as an urgent crisis, near-term effects have become more important, so many assessments and regulations have shifted toward using 20-year GWPs, with the explicit intention of prioritizing control of shorter-lived gases to reduce near-term heating.
Conclusions:
In conclusion, there are plenty of good reasons for the current priority focus on controlling methane. It’s a big source of global heating, second only to CO2 and contributing about a quarter of the current radiative forcing from long-lived greenhouse-gases. It’s increasing rapidly, and new scientific results are both showing its effect is bigger than previously thought – because emissions from human activities, especially from oil and gas operations, appear to have been substantially under-estimated – and also suggesting where and how to most effectively target controls, with increasing recognition of the large contributions from a few super-emitting sources and events.
This cuts both ways. On the one hand, continued expansion of natural-gas production at anything close to present leakage levels would be seriously harmful, probably putting climate goals like the Paris 1.5°C target definitively out of reach. Conversely, the near-term benefits of controlling methane can be very large. One recent study suggested that additional feasible methane cuts could eliminate 20 to 45 percent of the gap between present commitments and the Paris target, reducing heating in 2045 by nearly 0.3°C.
Finally, serious control of methane emissions appears to be technically tractable – because emissions are concentrated in a few types of activities that admit of technological controls. Moreover, many of these controls will be cheap. A recent study from the International Energy Agency estimates that three-quarters of present emissions from the oil and gas sector can be reduced with presently available technology, and that 40 percent of those cuts would carry zero or negative net cost. It’s ironic that that attractiveness of control arises because natural gas is a valuable fuel that the major emitters are already in the business of selling, but hey, I’ll take it. That also implies, of course, that the fraction of cuts that can be costless or profitable depends on the price of gas. The IEA also estimated that the big drop in gas prices that occurred during 2020 temporarily brought that no-net-cost fraction down from 40 percent to 10 percent.
This is all much-needed, relatively good news, but don’t be misled. All this well-deserved attention to the second-biggest source of global heating doesn’t mean that the imperative for strong action is reduced for the biggest source, CO2. Methane is one slice of the reduction pie, but the whole pie needs to be eaten. It’s lucky that methane controls are immediately available and relatively easy, but this is just a down-payment on the required total reduction
Reader Comments
2 Replies to “The Fuss about Methane”
Comments are closed.
Misses an important point. It’s always about the CO2 and going after methane is only important to the degree it degrade into CO2. Why?
https://hypergeometric.files.wordpress.com/2013/11/ghgabsorption.png
You three things to be a worrisome climate gas:
(1) Global Warming Potential, especially as a multiple of CO2’s GWP.
(2) Longevity in atmosphere. This can be somewhat relative when comparing short-lived climate pollutants with one another, but CO2 has a longevity of thousands of years.
(3) Earth’s outgoing thermal radiation has to cooperate. Yeah, CH4 has a theoretical GWP in its early life many times greater than CO2, but there is little outgoing thermal radiation from Earth in its absorption bands. In contrast, Earth’s 667 cm-1 bandis deep, wide, and corresponds to the biggest outgoing thermal band from Earth.
See the emission and absorption spectra in the figure above.
The global warming impact of methane [“natural” gas], as the author notes, is roughly estimated at about 25% of the global warming gas emissions, but does this Global Warming estimate include well known, documented, longstanding, massive, ongoing toxic emissions of methane by the politically powerful oil and gas industry, and also methane emissions from landfills and refineries which are allowed to emit or leak massive amounts of methane directly into the atmosphere?
Methane emissions are uncontrolled for one reason: industry has an army of cynical lobbyists and lawyers ‘working closely’ and with Congress, state Legislators, the senior EPA and state environmental officials to prevent regulation of methane leaks and emissions. Thus, landfills and refineries even when they capture methane are allowed to emit it uncontrolled, in massive amounts, every single day.
The Bush 43, Obama and Trump Administrations and all the states and EPA Administrators for all these decades have rejected citizen comments and pleadings to require methane controls and preventive measures.
The lobbyists for the industry are winning fossil fuel subsidies and avoiding methane limits and controls, it does not matter that controls are cheap, cost effective, and unequivocally necessary to protect public health and the environment.